-
oa Platinum Metals as Hydrogenation Catalysts
- Source: Platinum Metals Review, Volume 1, Issue 3, Jul 1957, p. 87 - 93
-
- 01 Jan 1957
Preview this article:
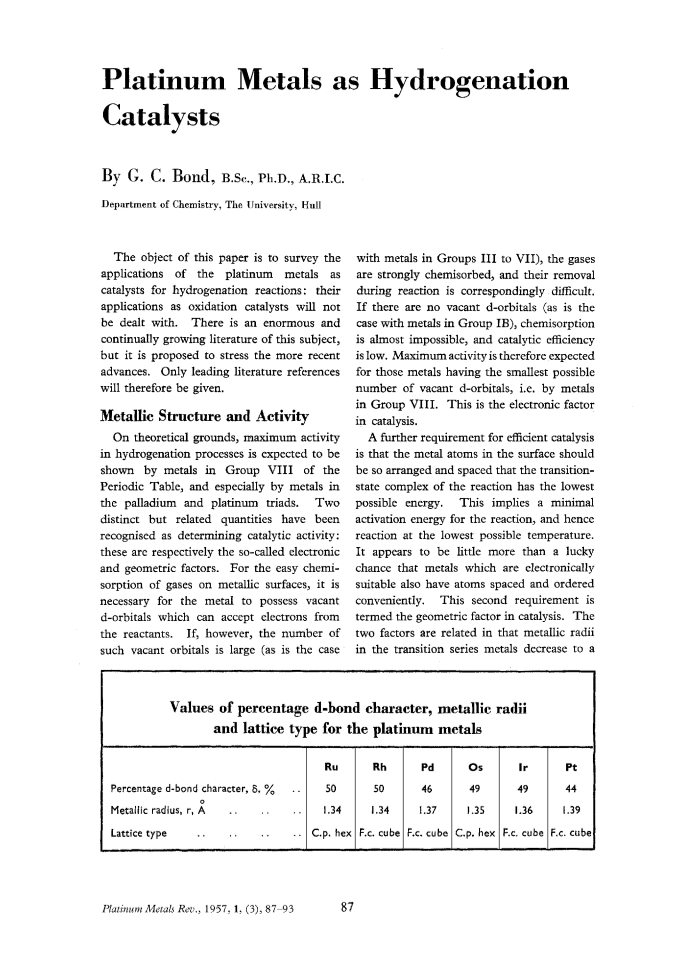



Platinum Metals as Hydrogenation Catalysts, Page 1 of 1
< Previous page Next page > /docserver/preview/fulltext/pmr/1/3/pmr0001-0087-1.gif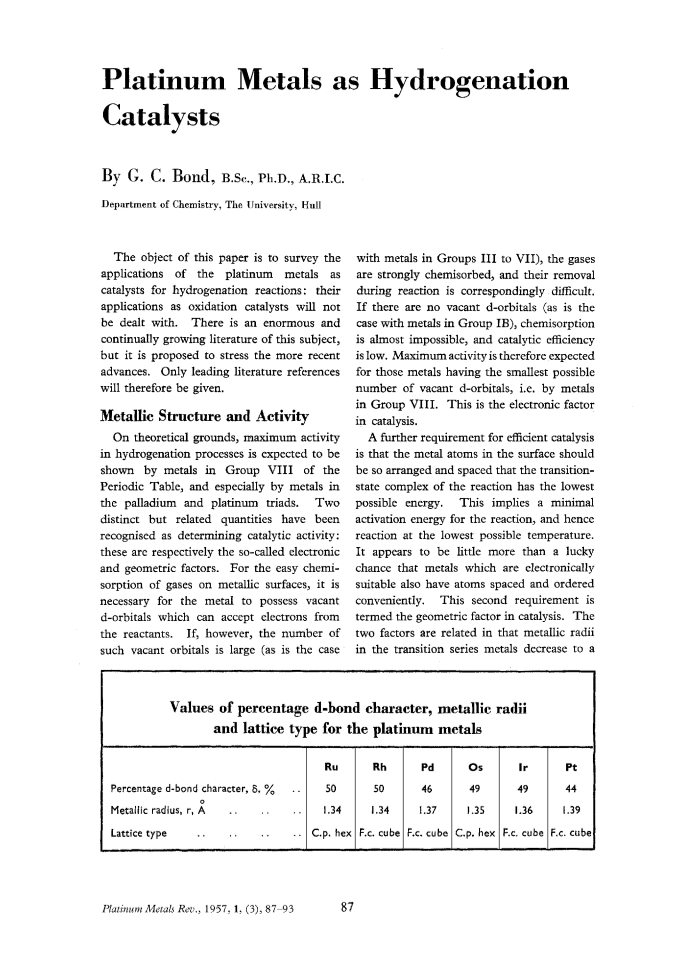
There is no abstract available.
© Johnson Matthey