-
oa Wear and Friction of the Platinum Metals
Performance in Sliding Contacts
- Source: Platinum Metals Review, Volume 10, Issue 1, Jan 1966, p. 2 - 8
-
- 01 Jan 1966
Preview this article:
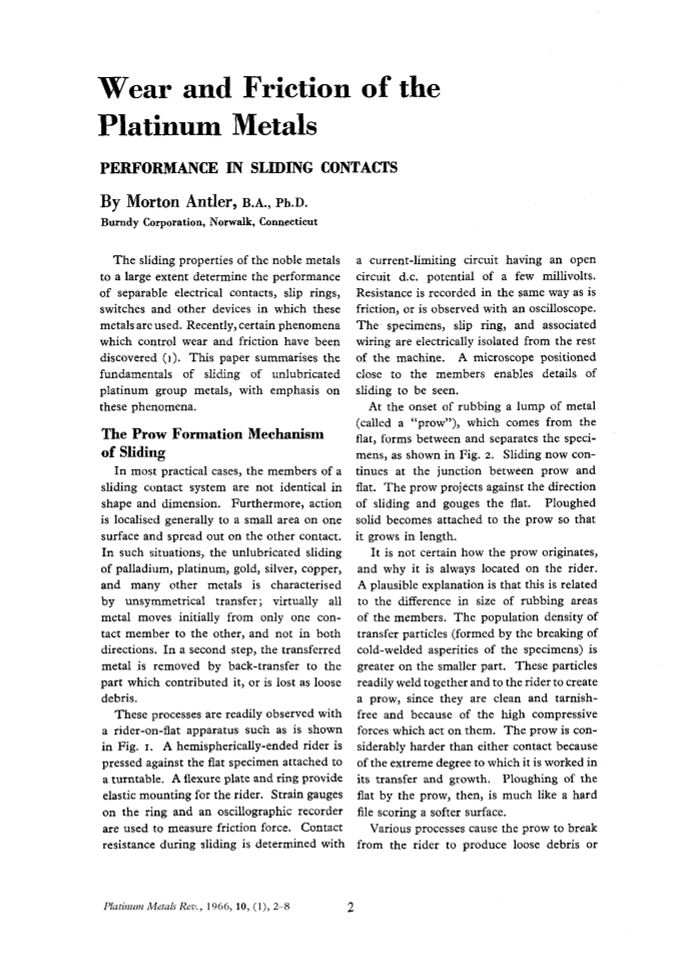



Wear and Friction of the Platinum Metals, Page 1 of 1
< Previous page Next page > /docserver/preview/fulltext/pmr/10/1/pmr0010-0002-1.gif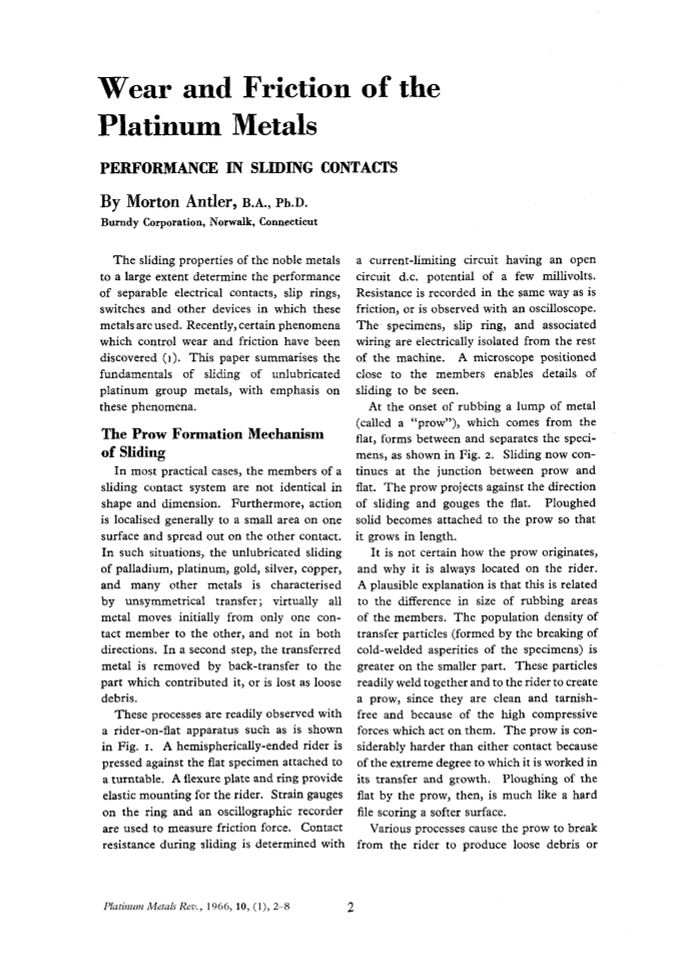
There is no abstract available.
© Johnson Matthey