-
oa The Alloying of Titanium Aluminides with Ruthenium
- Source: Platinum Metals Review, Volume 33, Issue 3, Jul 1989, p. 106 - 113
-
- 01 Jan 1989
Preview this article:
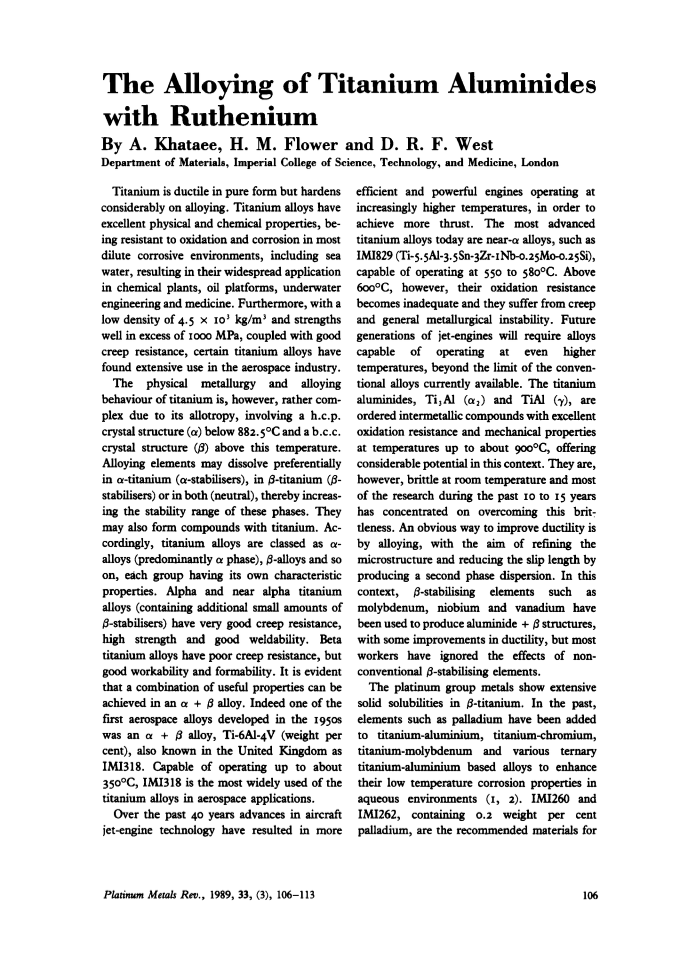



The Alloying of Titanium Aluminides with Ruthenium, Page 1 of 1
< Previous page Next page > /docserver/preview/fulltext/pmr/33/3/pmr0033-0106-1.gif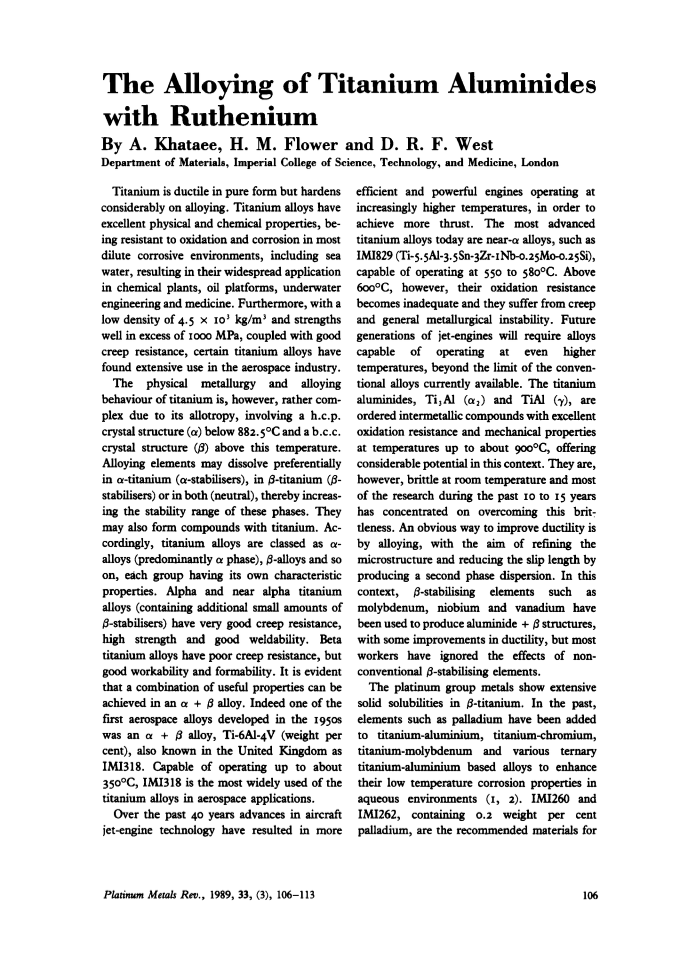
There is no abstract available.
© Johnson Matthey