-
oa “Organometallics as Catalysts in the Fine Chemical Industry”
- Source: Platinum Metals Review, Volume 57, Issue 4, Oct 2013, p. 272 - 280
Preview this article:
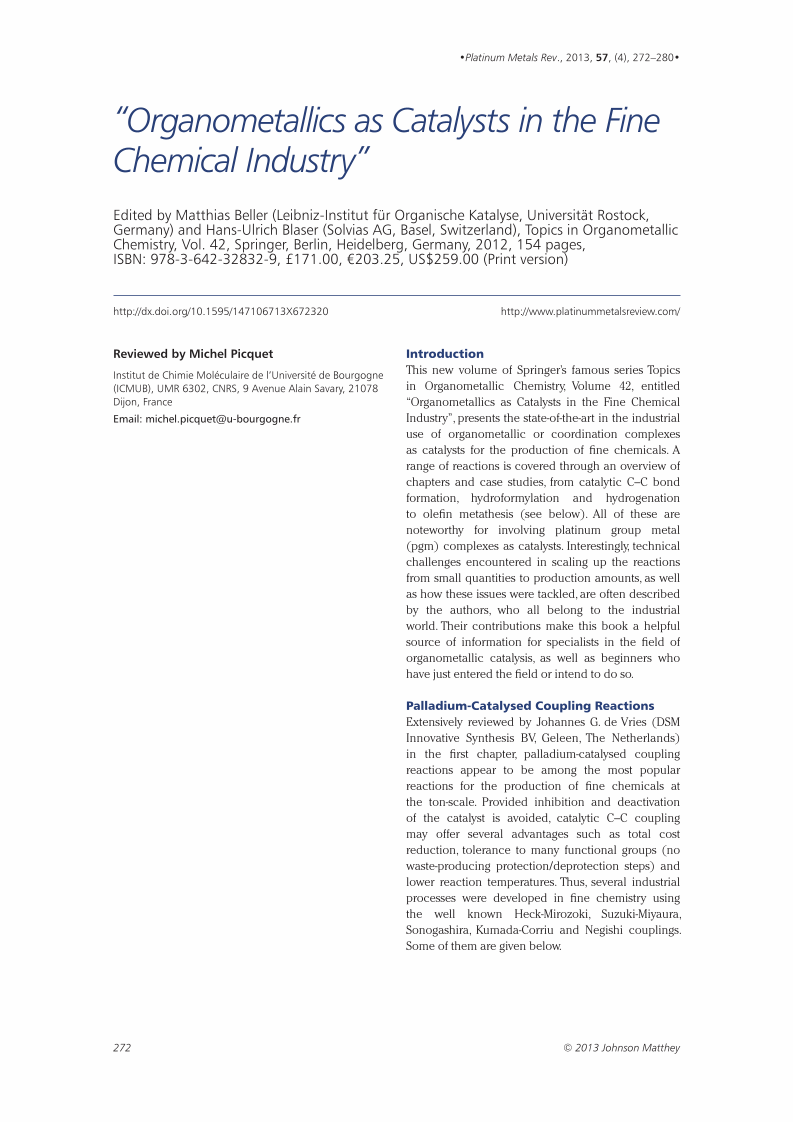



“Organometallics as Catalysts in the Fine Chemical Industry”, Page 1 of 1
< Previous page Next page > /docserver/preview/fulltext/pmr/57/4/s3-1.gif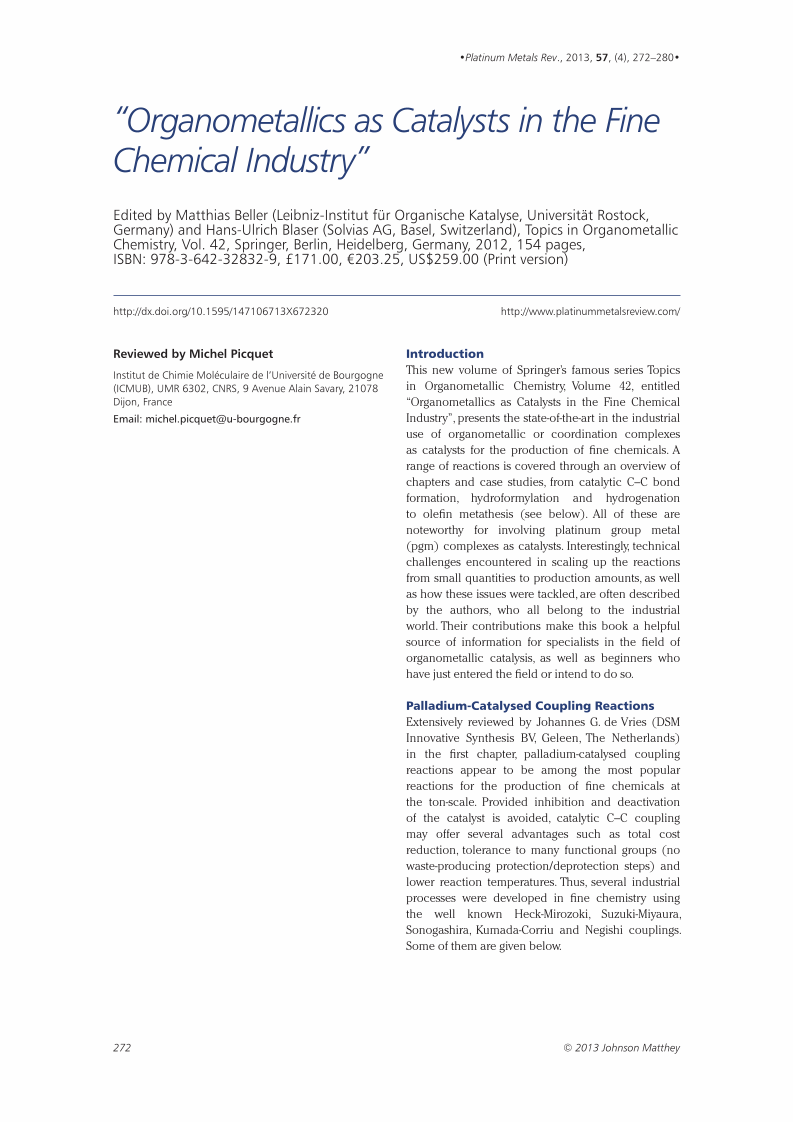
There is no abstract available.
© Johnson Matthey