-
oa FINAL ANALYSIS: Characterisation of Catalysts Using Secondary and Backscattered Electron In-lens Detectors
- Source: Platinum Metals Review, Volume 58, Issue 2, Apr 2014, p. 106 - 110
-
- 01 Jan 2014
Preview this article:
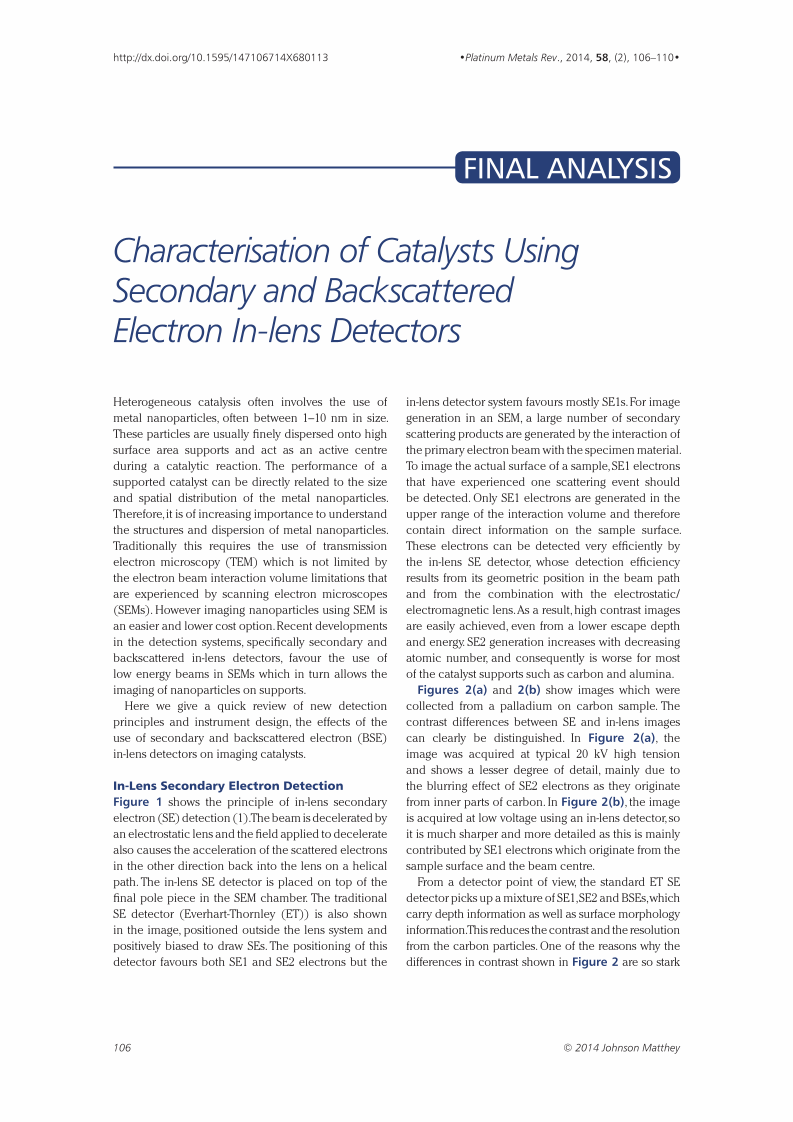



FINAL ANALYSIS: Characterisation of Catalysts Using Secondary and Backscattered Electron In-lens Detectors, Page 1 of 1
< Previous page Next page > /docserver/preview/fulltext/pmr/58/2/PMR-58-2-Kuo-1.gif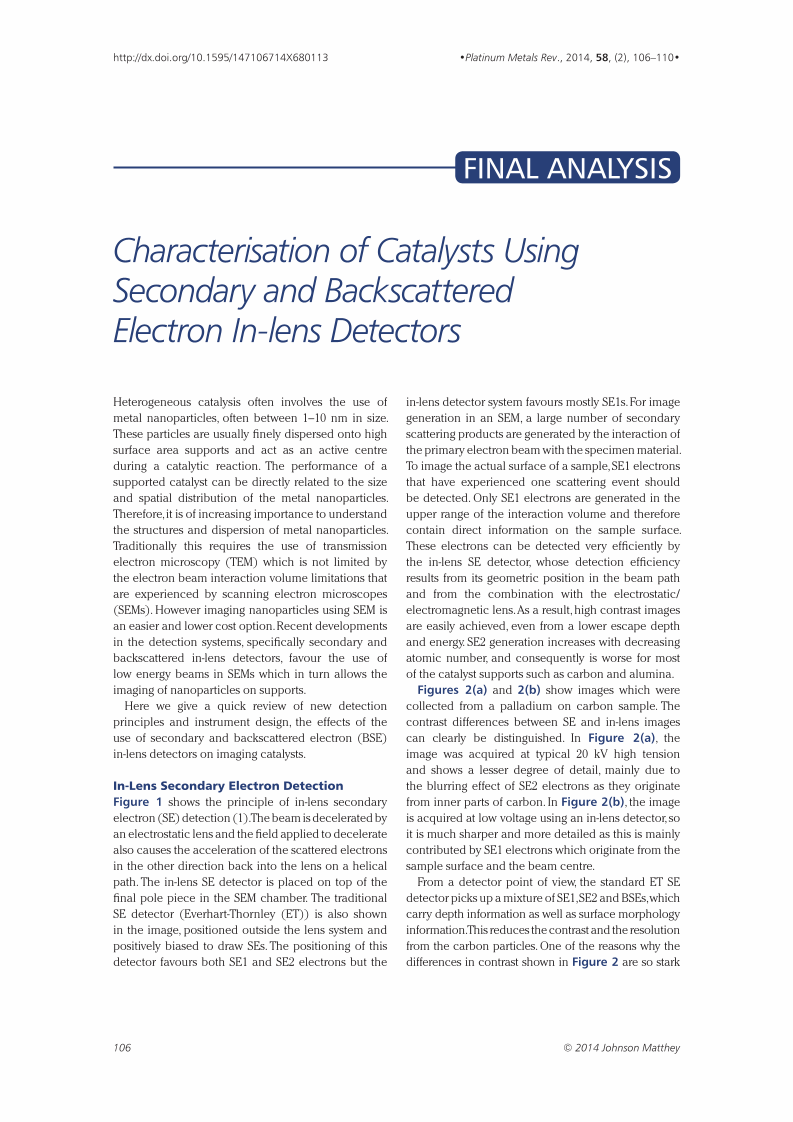
There is no abstract available.
© Johnson Matthey