-
oa Deformation of Zone-melted Iridium Single Crystals
- Source: Platinum Metals Review, Volume 8, Issue 3, Jul 1964, p. 102 - 106
-
- 01 Jan 1964
Preview this article:
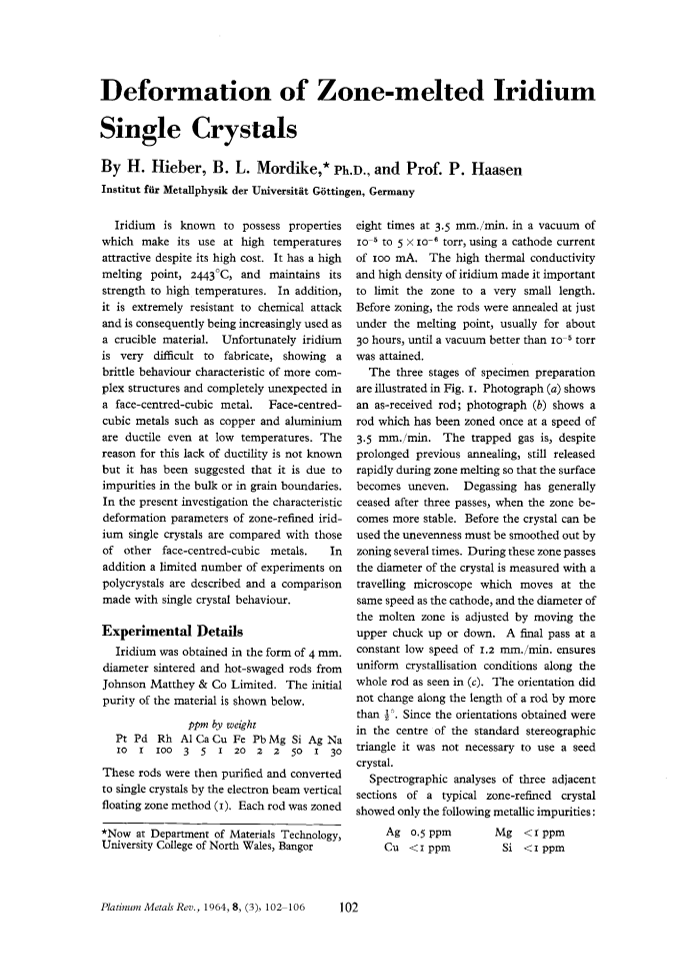



Deformation of Zone-melted Iridium Single Crystals, Page 1 of 1
< Previous page Next page > /docserver/preview/fulltext/pmr/8/3/pmr0008-0102-1.gif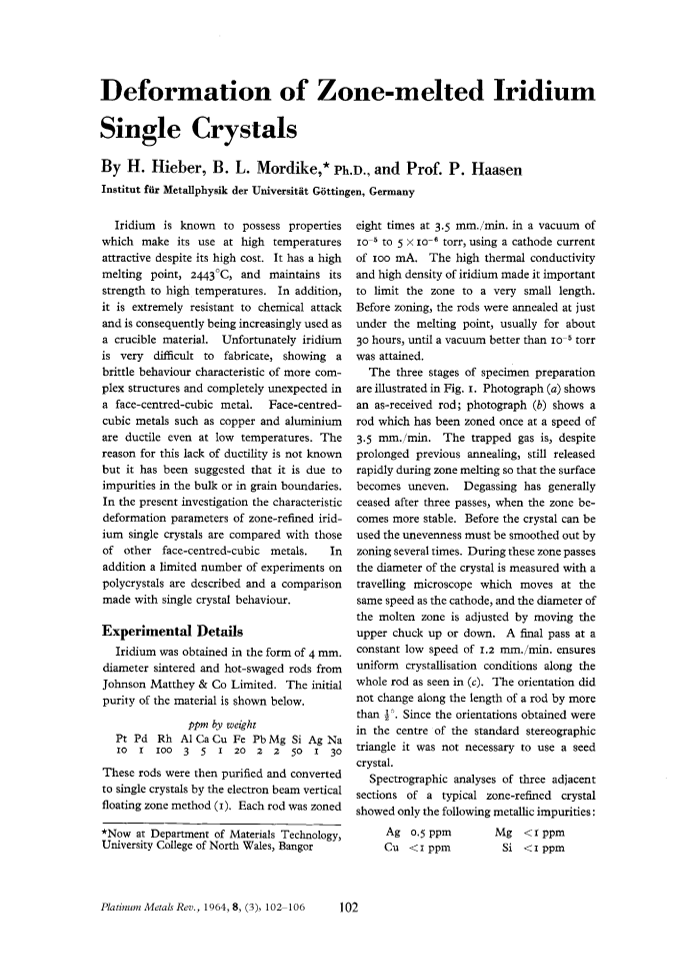
There is no abstract available.
© Johnson Matthey