-
oa 12th Greenhouse Gas Control Technologies Conference
- Source: Johnson Matthey Technology Review, Volume 59, Issue 3, Jul 2015, p. 182 - 187
Preview this article:
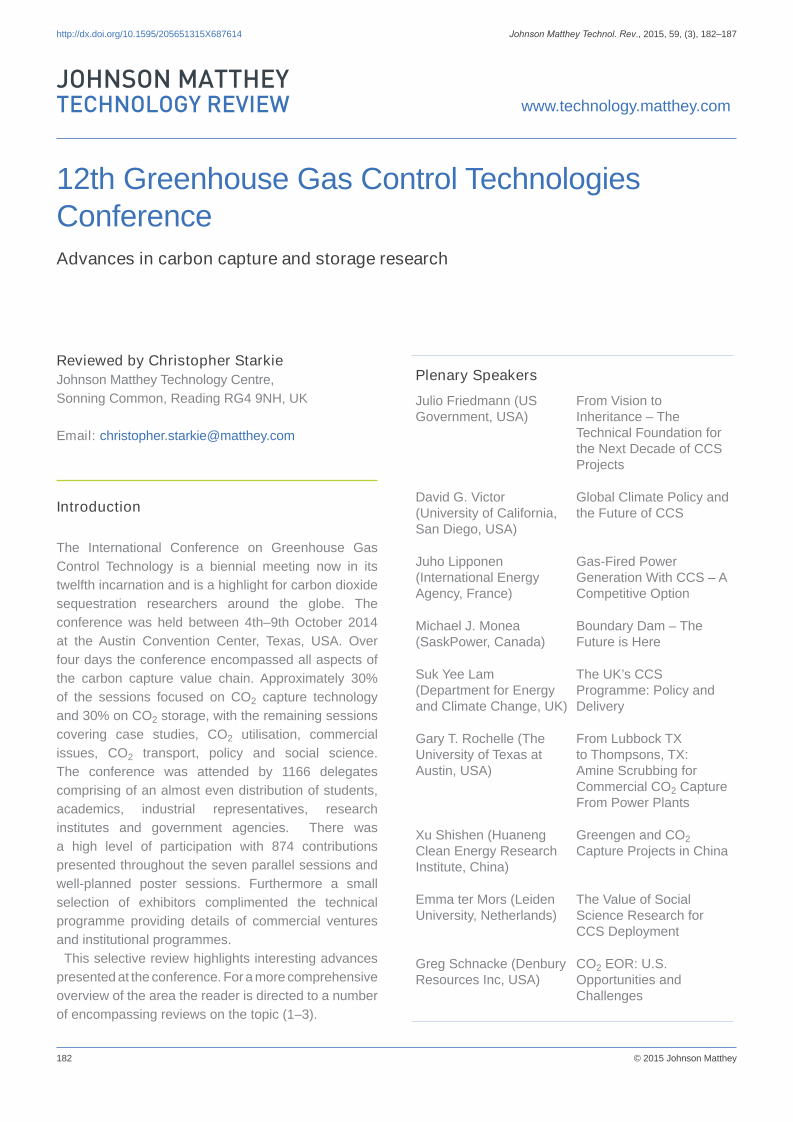



12th Greenhouse Gas Control Technologies Conference, Page 1 of 1
< Previous page Next page > /docserver/preview/fulltext/pmr/59/3/s2-1.gif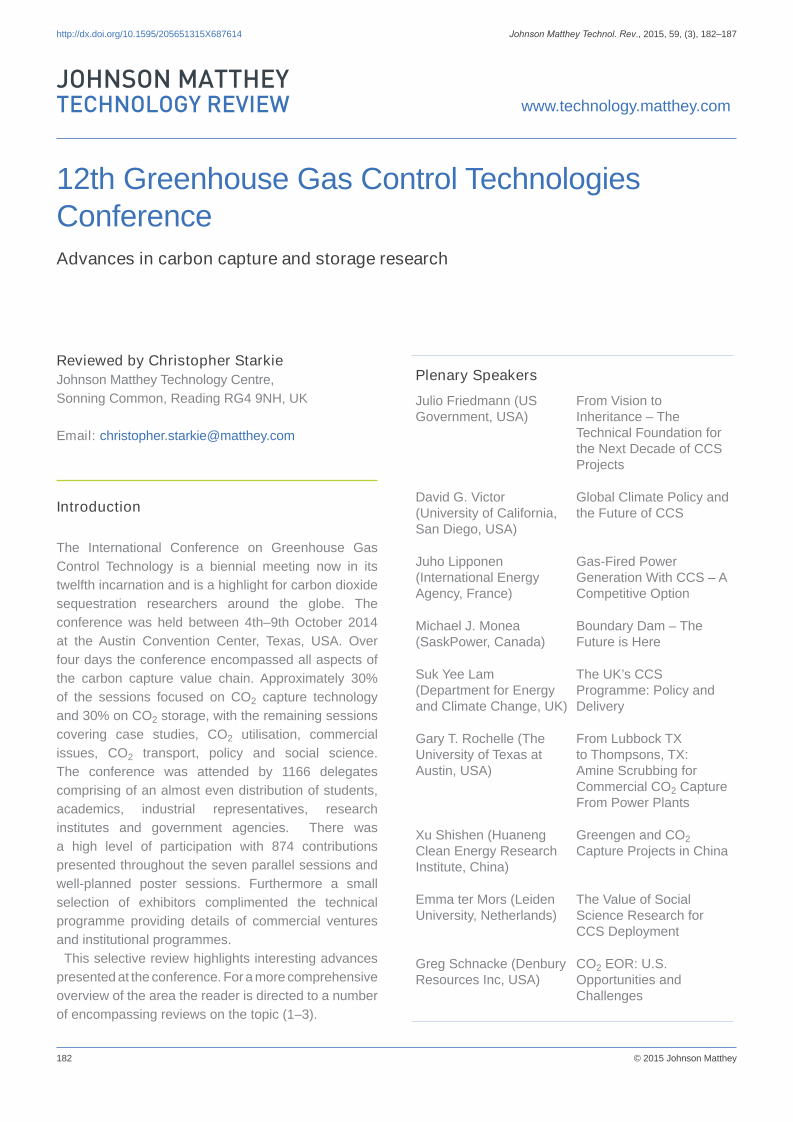
There is no abstract available.
© Johnson Matthey