-
oa Carbon Formation in Steam Reforming and Effect of Potassium Promotion
Potassium dopants prevent carbon formation and aid catalyst recovery
- Source: Johnson Matthey Technology Review, Volume 59, Issue 4, Oct 2015, p. 313 - 318
-
- 01 Jan 2015
Preview this article:
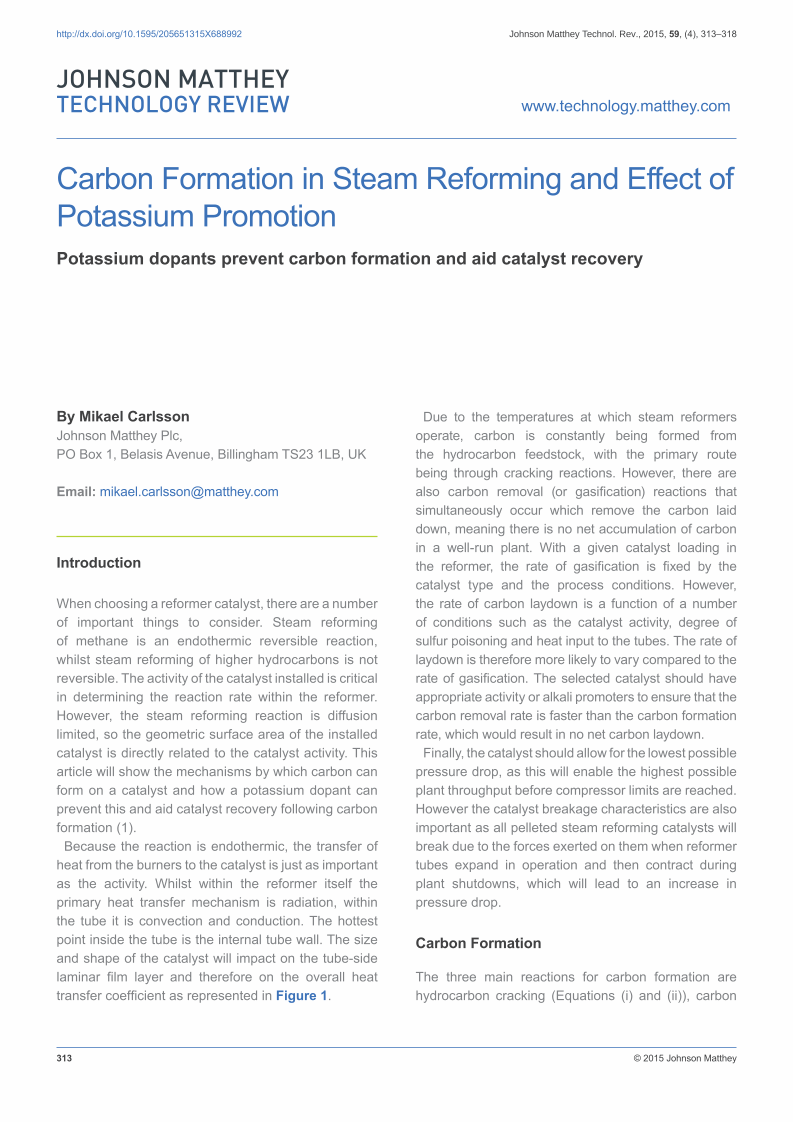



Carbon Formation in Steam Reforming and Effect of Potassium Promotion, Page 1 of 1
< Previous page Next page > /docserver/preview/fulltext/jmtr/59/4/JMTR-59-4-Carlsson-1.gif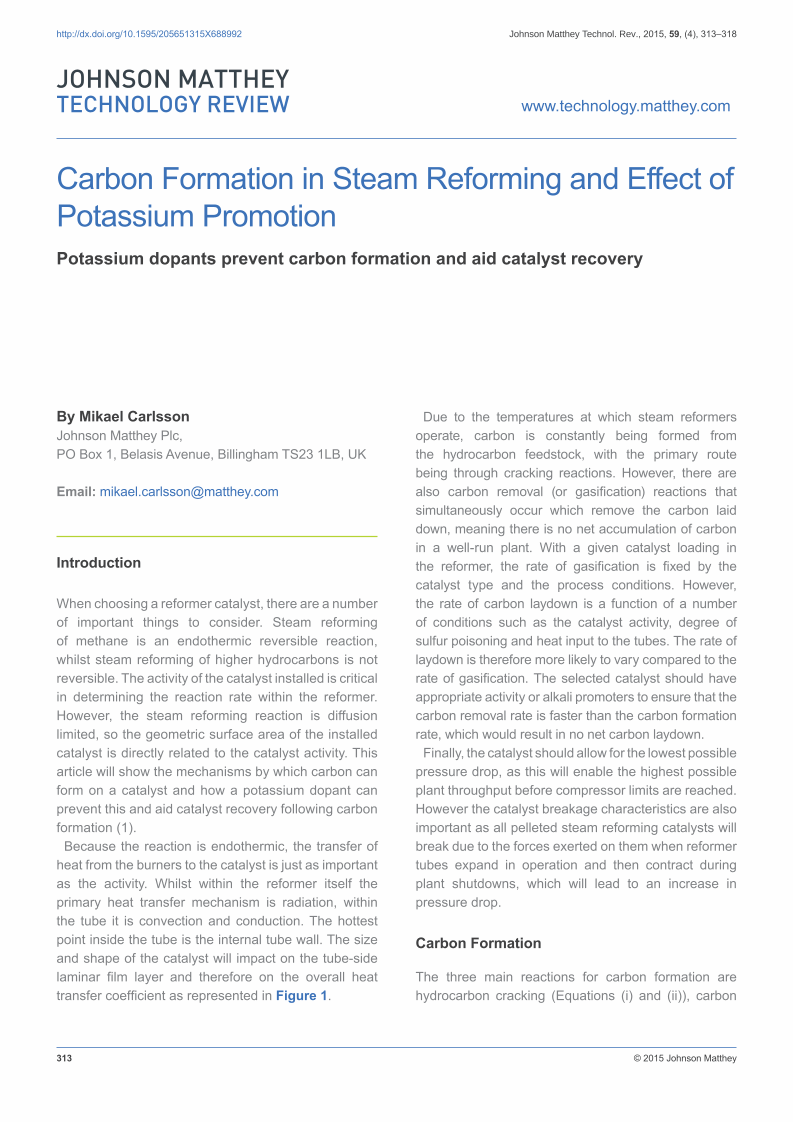
There is no abstract available.
© Johnson Matthey