-
oa Selective Removal of Mercury from Gold Bearing Streams
Exploring the use of solid adsorbents to avoid the undesirable loss of gold
- Source: Johnson Matthey Technology Review, Volume 59, Issue 4, Oct 2015, p. 322 - 330
-
- 01 Jan 2015
Preview this article:
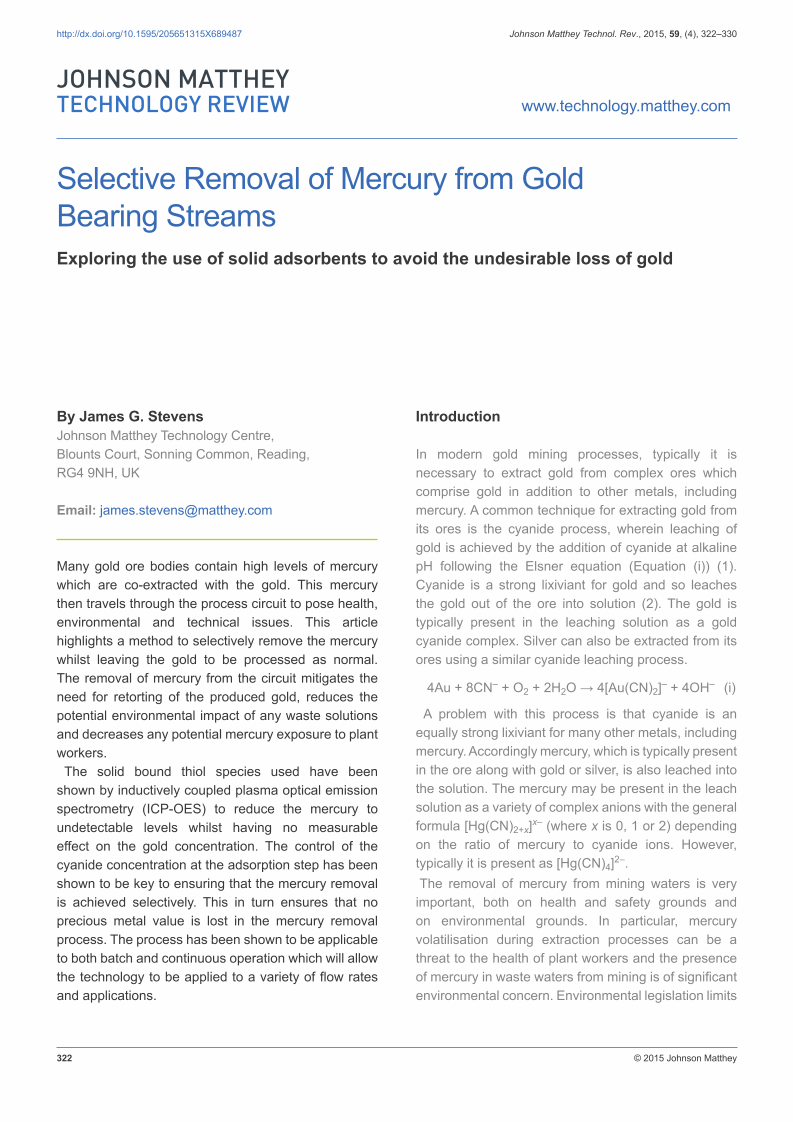



Selective Removal of Mercury from Gold Bearing Streams, Page 1 of 1
< Previous page Next page > /docserver/preview/fulltext/jmtr/59/4/JMTR-59-4-Stevens-1.gif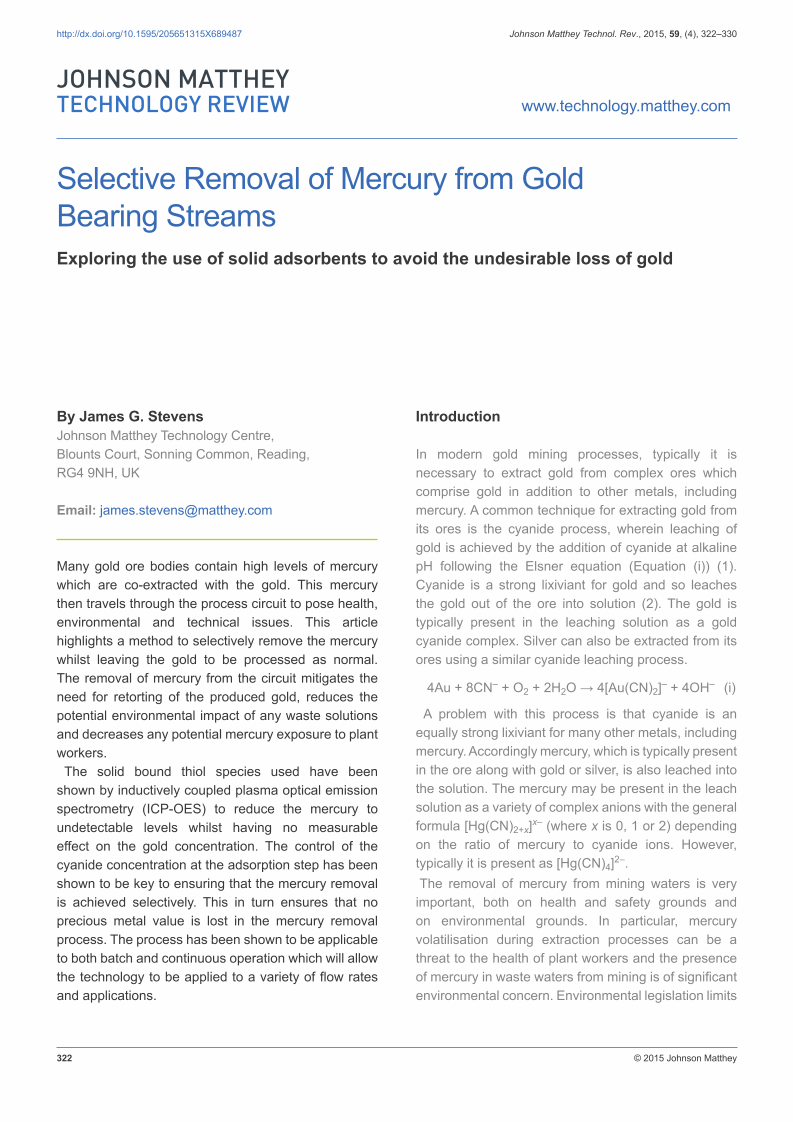
There is no abstract available.
© Johnson Matthey