-
oa In the Lab: Bespoke Green Nanomaterials: Discovery, Design, Applications and Manufacture
Johnson Matthey Technology Review features new laboratory research
- Source: Johnson Matthey Technology Review, Volume 63, Issue 3, Jul 2019, p. 152 - 156
-
- 01 Jan 2019
Preview this article:
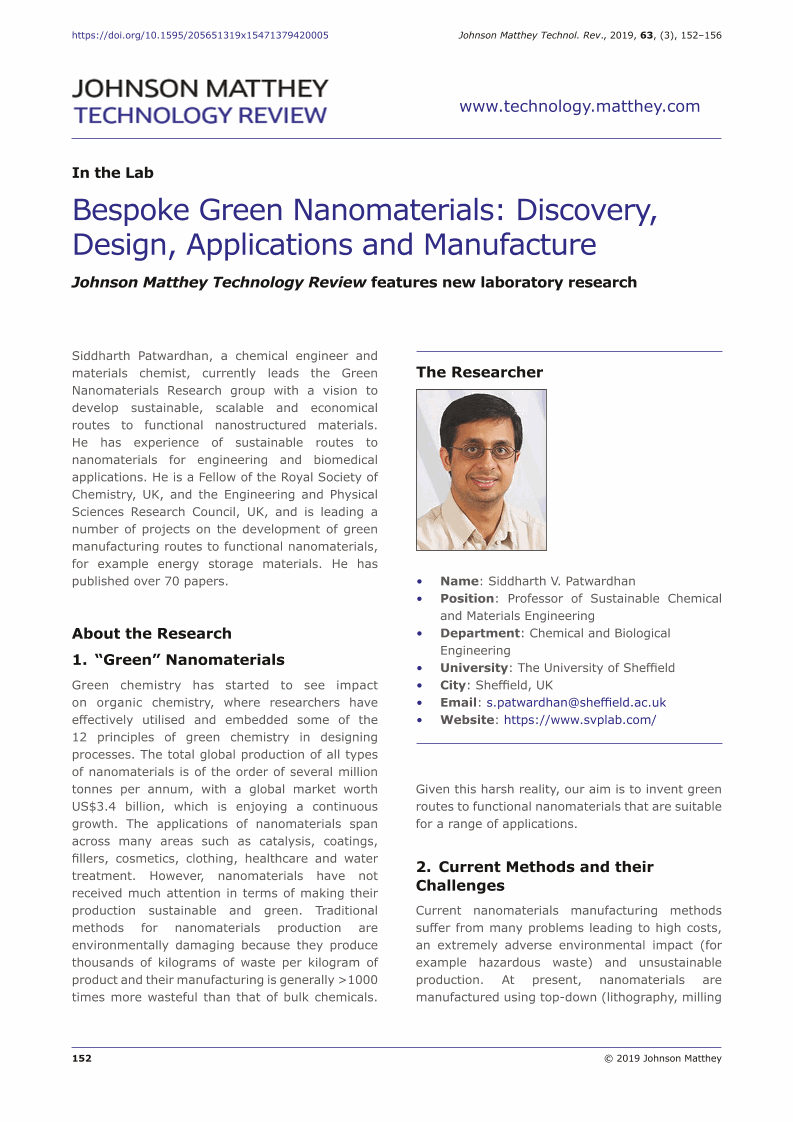



In the Lab: Bespoke Green Nanomaterials: Discovery, Design, Applications and Manufacture, Page 1 of 1
< Previous page Next page > /docserver/preview/fulltext/jmtr/63/3/Patwardhan_16a_Imp-1.gif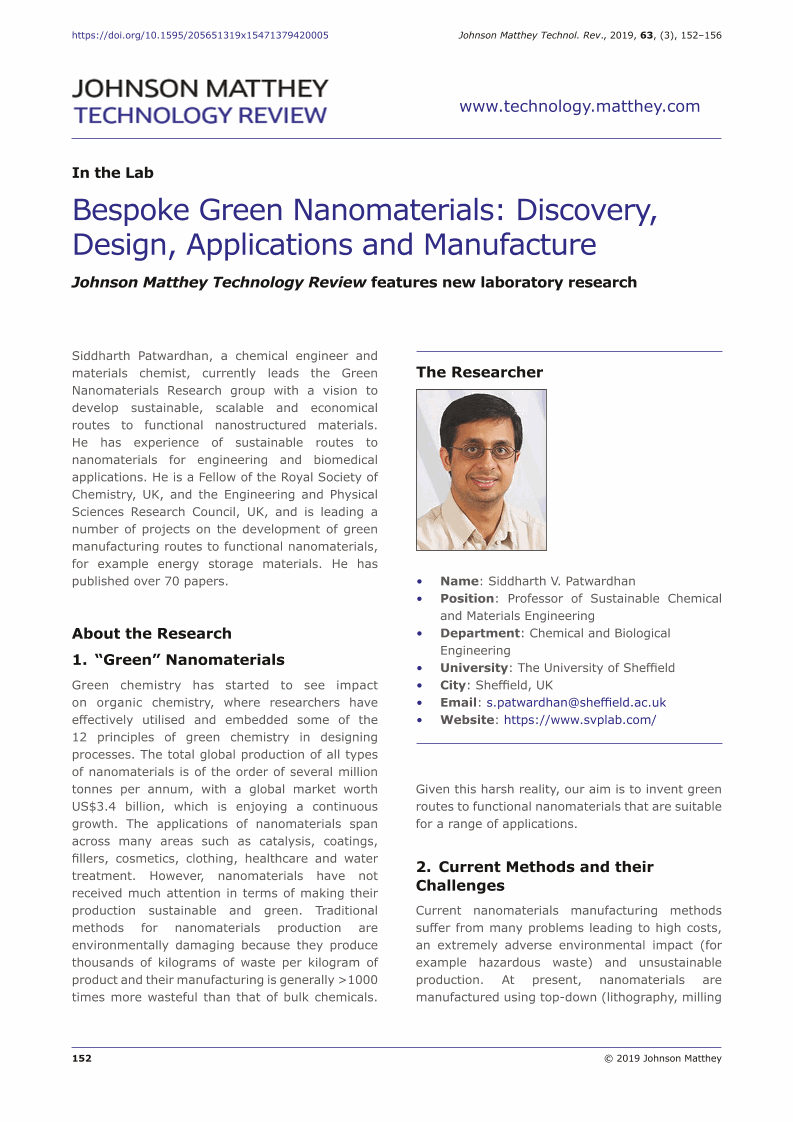
There is no abstract available.
© Johnson Matthey