-
oa “Nanocarbons for Energy Conversion: Supramolecular Approaches”
Edited by Naotoshi Nakashima (Kyushu University, Japan), Nanostructure Science and Technology, Springer International Publishing AG, part of Springer Nature, Switzerland, 2019, 564 pp, ISBN: 978-3-319-92915-6, £129.99, €160.49, US$179.99
- Source: Johnson Matthey Technology Review, Volume 63, Issue 4, Oct 2019, p. 281 - 284
-
- 01 Jan 2019
Preview this article:
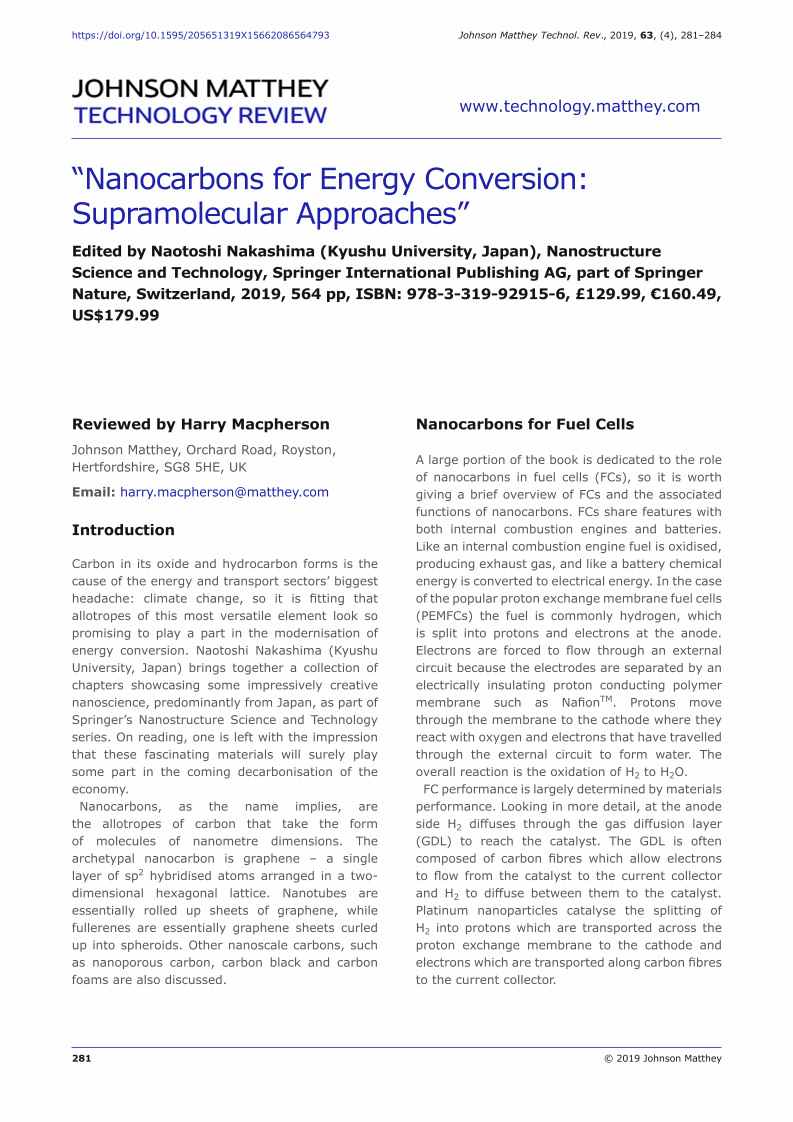



“Nanocarbons for Energy Conversion: Supramolecular Approaches”, Page 1 of 1
< Previous page Next page > /docserver/preview/fulltext/jmtr/63/4/Macpherson_16a_Imp-1.gif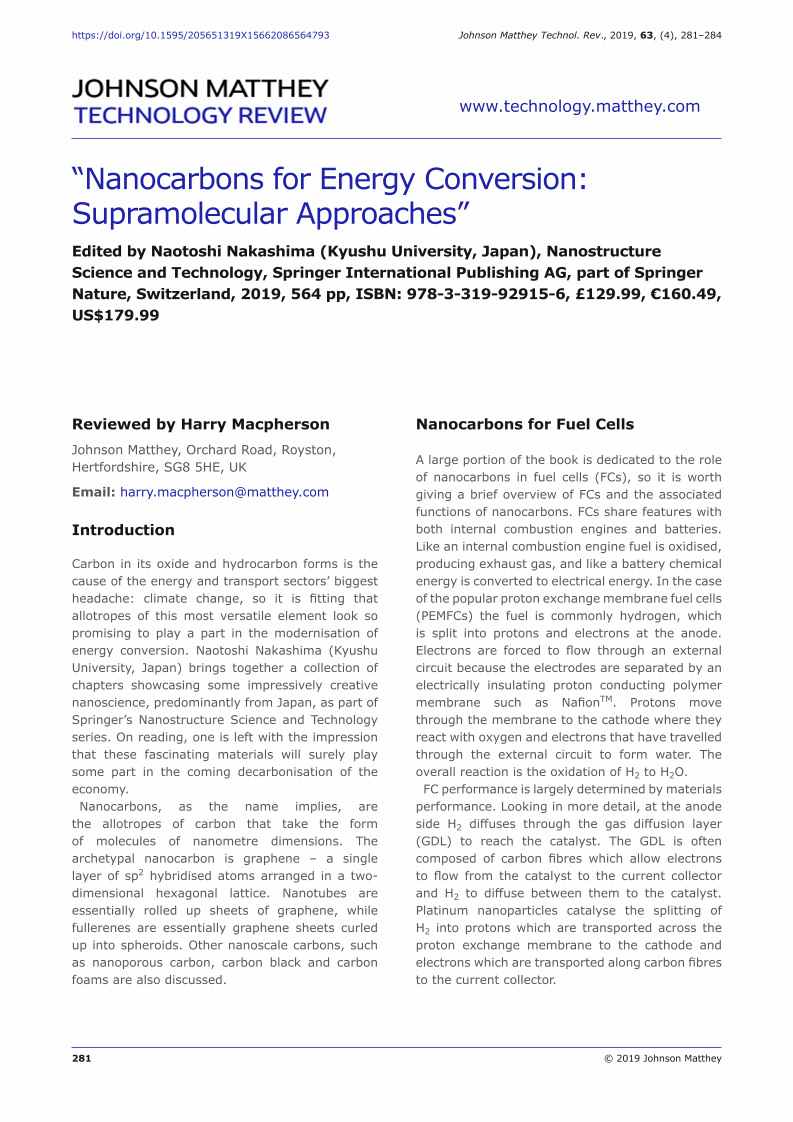
There is no abstract available.
© Johnson Matthey