-
oa 14th Ulm Electrochemical Talks
Towards next generation technologies for a sustainable electric future
- Source: Johnson Matthey Technology Review, Volume 59, Issue 2, Apr 2015, p. 90 - 94
-
- 01 Jan 2015
Preview this article:
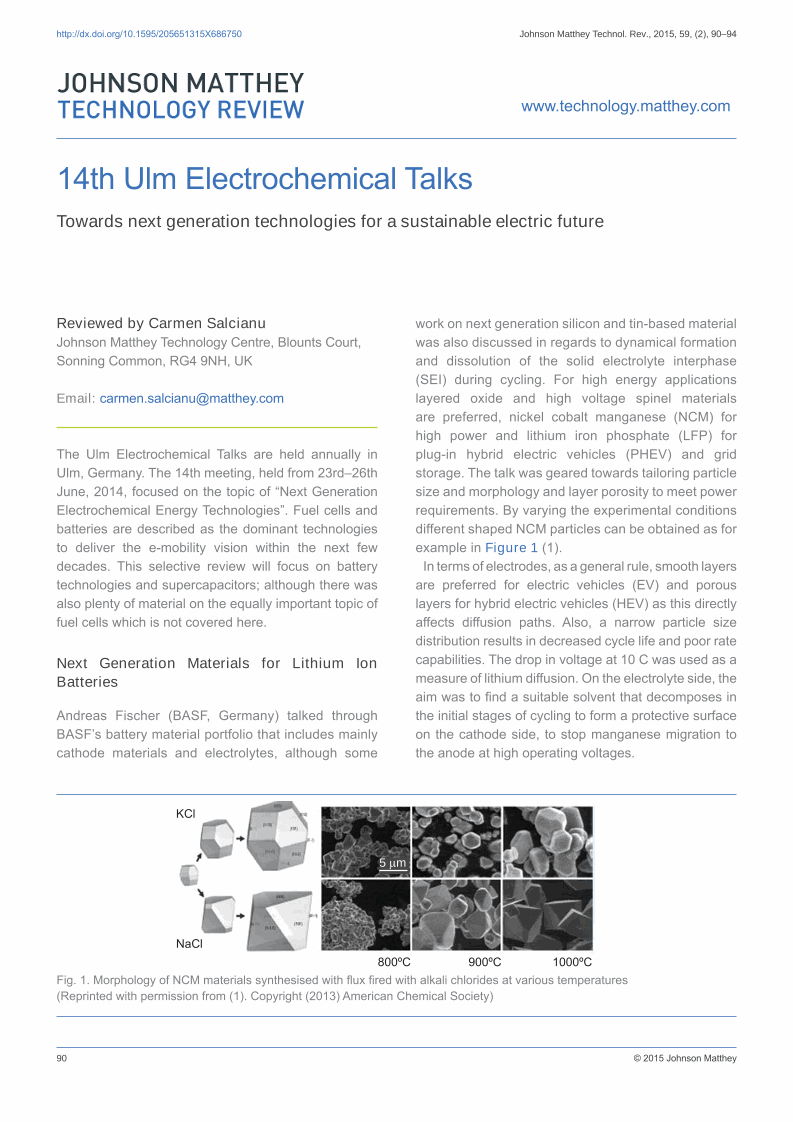



14th Ulm Electrochemical Talks, Page 1 of 1
< Previous page Next page > /docserver/preview/fulltext/jmtr/59/2/JMTR-59-2-Salcianu-1.gif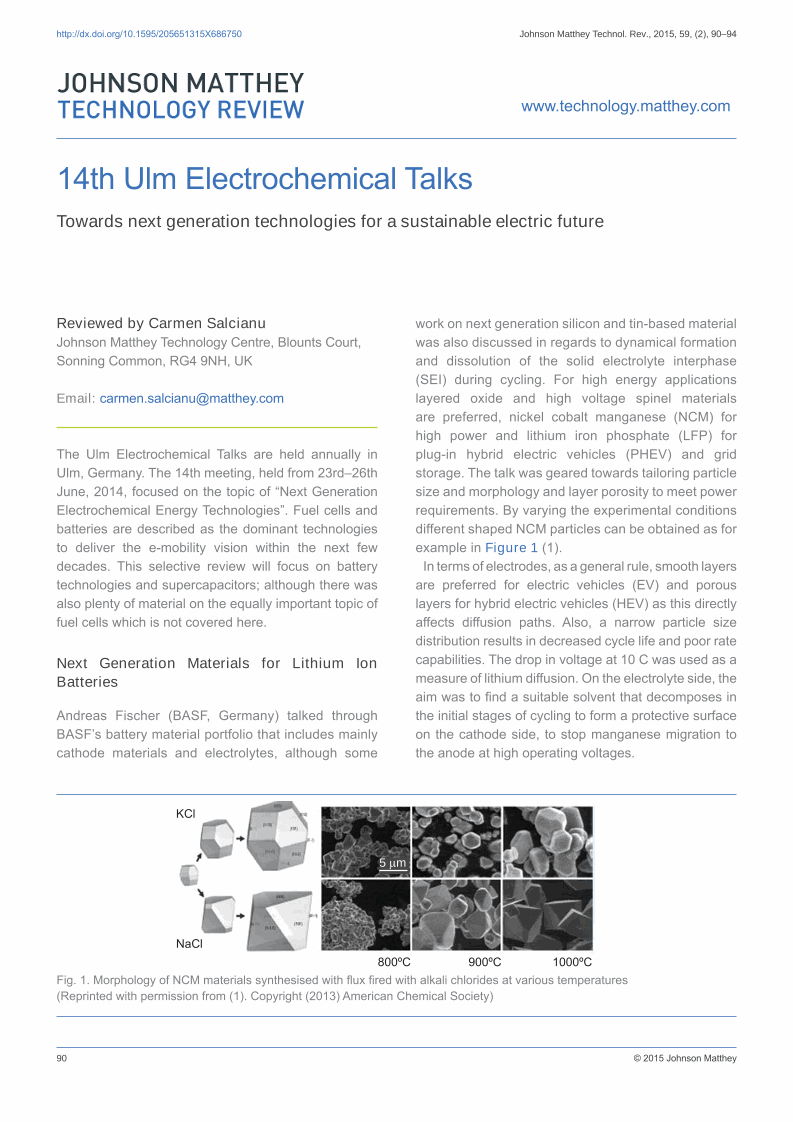
There is no abstract available.
© Johnson Matthey